Capturing the Ghosts of the Universe
70 Billion. That’s how many particles are passing through every square centimetre of your skin each second. This is not a sci-fi novel; the most fundamental laws of the universe conspire to make it so.
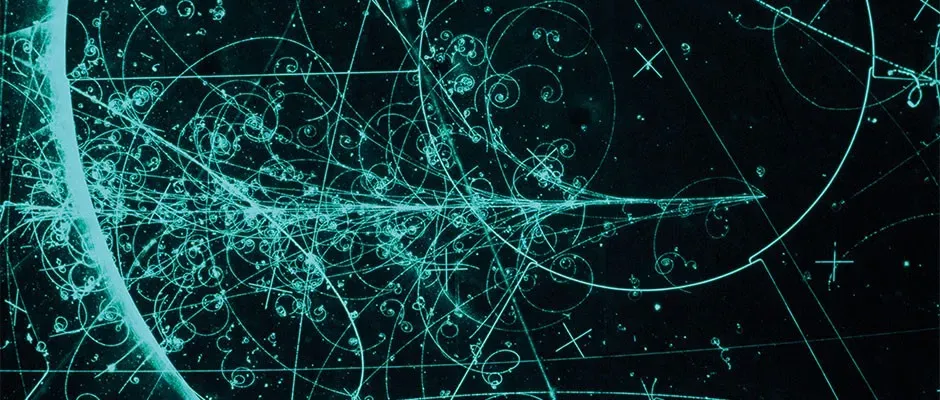
70 Billion [1]. That’s how many particles are passing through every square centimetre of your skin each second. This is not a sci-fi novel; the most fundamental laws of the universe conspire to make it so.
The particle in question is the neutrino. Predicted by Pauli in 1930, whose investigations of radioactive Beta-decay suggested the existence of the emission of a second, undetected particle along with the electron [2], the neutrino comes in three “flavours”: the electron, muon and tau neutrinos. Virtually massless, chargeless, and unbound by the strong nuclear force of the quarks, the neutrinos are reluctant to interact with anything, only doing so significantly via the weak interaction. Indeed, it is said that “a neutrino of moderate energy could easily penetrate a thousand light-years of lead(!)” [3].
It is these properties which make neutrinos an ideal subject for astronomic observation. Being chargeless, neutrinos are unaffected by deflection from galactic magnetic fields which could obscure their source. They are also fundamental, meaning they do not decay between their source and detectors on Earth, and their famous penetrative ability means that they can both be detected through otherwise obscuring galactic dust and from the interior of astronomical bodies [1].
In order to use neutrinos for astronomy, of course, one must actually be able to detect them, and with the notoriously prudish nature of the neutrino when it comes to interactions, the instruments we use to observe them are triumphs of experimental design.
Solar Mysteries
The first astrophysical neutrino detector was built in the Homestake Gold mine in South Dakota by Raymond Davies Jr [4]. Going online in 1969, the detector consisted of a large vat of perchloroethylene [5], essentially cleaning fluid. When a neutrino interacts with an atom of Chlorine-37 (an isotope of chlorine which makes up ~25% of naturally occurring chlorine on earth [6]), a neutron in the chlorine nucleus changes into a proton, forming Argon-37[7], which is released into the fluid as a gas. After a period of time, the amount of argon in the chamber is measured, and the number of neutrino detections can be calculated [5].
Modern astrophysical neutrino detectors, namely the Sudbury Neutrino Observatory (SNO), Super-Kamiokande and IceCube detectors function on a somewhat different principle. All three use water in some form: 1000 tonnes of heavy water [8], 50,000 tons of water [4] and 1 cubic kilometre of Antarctic ice [9] respectively. The lepton produced by the interaction of neutrinos with the protons in the water molecules is detected through emission of Cherenkov radiation. Since this radiation is emitted at a very predictable angle and frequency, the path of the neutrino through the detector can be reconstructed [10] (See Figure 1), along with the energy of the neutrino.

The first object to be observed in neutrinos was the closest known source: the Sun. The Sun produces neutrinos through several different processes, all of which produce only electron neutrinos [5]. The most significant of these is the so-called “pp-chain” involving the fusion of two protons [5]. The Homestake detector’s Chlorine-37 interaction, however, was not sensitive to neutrinos from this chain due to their low energy, and so it was neutrinos from other chains – most notably Boron-8 decay — which were detected [5]. Indeed, neutrinos from the pp-chain were not directly detected until as late as 2014 [11]!
The observation of solar neutrino flux is useful for investigating the structure of the solar core, since the reactions which produce neutrinos are highly dependent on temperature. The neutrino flux from Boron-8 decay varies particularly strongly, with dependence claimed variously from T¹⁸ [1] to as much as T²⁴ [5]. As such, we see in Figure 2 that the rate of neutrino production from the Boron-8 decay chain quickly goes to zero outside 0.1 solar radii. By measuring the flux of these neutrinos, we can study the temperature variations within the core of our local star [5].
It was swiftly noticed, however, that the detection rate of electron neutrinos in the Homestake detector was almost a quarter of that which would be predicted from the best solar and particle physics models of the day. The prediction was a Boron-8 neutrino detection rate of of 7.9 ± 2.1 SNU (Solar Neutrino Unit), while the observed detection rate was 2.1 ± 0.9 SNU, well outside of the uncertainty range of the prediction [5]. There were numerous suggestions for solutions to this “Solar Neutrino Problem”, ranging from the sensible idea that we may just misunderstand an aspect of stellar dynamics [5], to the outright strange notion that the disparity was a sign of the shutting down of solar fusion altogether [1]!

The explanation, as it turned out, was neutrino oscillations. This is the idea that the neutrino flavours are different superpositions of quantum mass eigenstates, and, just as a superposition of energy eigenstates oscillates back and forth in space over time, neutrinos oscillate back and forth in flavour with time [4]. Thus, a proportion of the electron neutrinos produced in the sun will have oscillated into one of the other flavours by the time they reach Earth and will not be detected in an instrument sensitive only to electron neutrinos, such as the Homestake Mine Detector. Thus, the observed neutrino flux will be less than predicted [12].
The existence of neutrino oscillations was confirmed by results from the Super-Kamiokande detector in the late 1990s [4], and their role in the Solar Neutrino Problem was confirmed in 2001 by the collaboration at the SNO [12]. More importantly, the existence of neutrino oscillations confirms that the neutrino has mass, a Standard Model-defying conclusion, which won Takaaki Kajita of Super-Kamiokande and Arthur B. McDonald of the SNO the 2015 Nobel prize for physics [13].
Noisy Neighbours
The resolution of the Solar Neutrino Problem was, as is much of science, the result of decades of collaboration. 1987, however, saw the first notable single event in neutrino astrophysics. In February of that year, the blue supergiant star Sanduleak, located about 170,000 light years from Earth in the Large Magellanic Cloud [1], went supernova [14]. Designated SN1987A, an image of the remnants of the event can be seen in Figure 3.
Supernovae produce neutrinos in two main ways. The first, known as deleptonisation, occurs during the final stages of core collapse. This is where the core of the star becomes so compressed that electrons are forced into the nucleus of atoms, where they combine with protons to form neutrons. The process therefore requires the release of an electron neutrino to conserve lepton number [1].
The second process occurs during the immense explosion which follows core collapse, as thermal photons of energies up to 10 MeV form electron-positron pairs, which combine to form neutral Z bosons. These then decay into neutrino-antineutrino pairs, producing all three flavours of neutrino with equal probability [1].
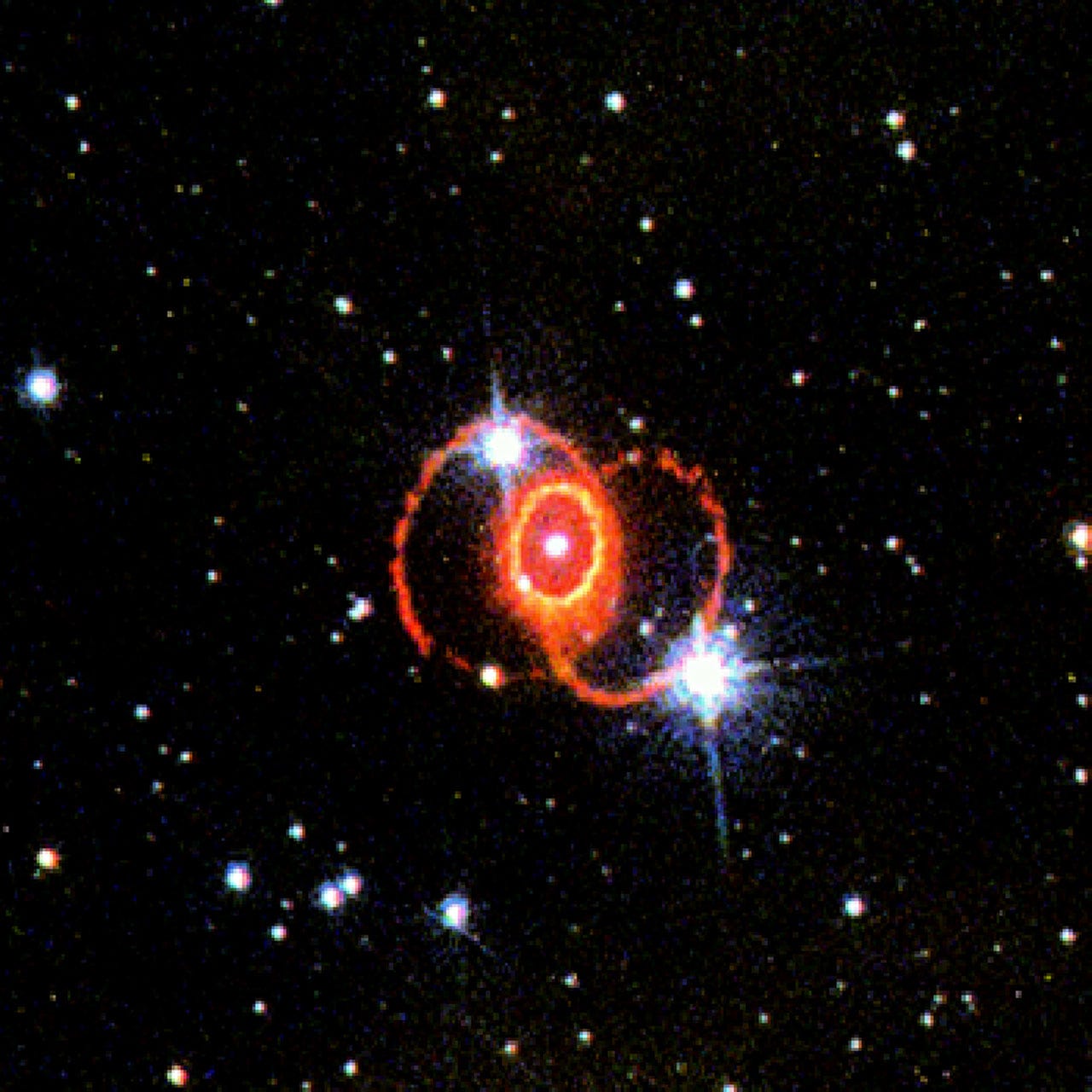
An estimated 10⁵⁸ neutrinos [1] were emitted during SN1987A, of which 20 were detected between the Kamiokande-II (a predecessor of Super-Kamiokande) and Irvine–Michigan–Brookhaven detectors. The first detections at each site were simultaneous “to within the accuracy of the known zero point” [5], which indicated that the detections were extremely likely to be connected. The near simultaneity with the optical observation of the supernova [14] and the similarity of the detections to those which would be expected for a type II supernova such as this, suggested that the neutrino burst was associated with SN1987A. As such, the detections amount to “the first direct observation in neutrino astronomy” [15].
While the supernova provided evidence that our understanding of one of the universe’s most energetic events is accurate, it was again the knowledge gained about the neutrino itself which proved especially insightful. One of the key results was the fact that neutrinos of different energies arrived at different times. A consequence of special relativity is that, regardless of energy, any massless particle must propagate at the speed of light. The apparent dependence between energy and arrival time indicated that the neutrinos, while travelling very close to the speed of light, were not travelling at the speed of light, which indicated that the neutrino ought to have at least some mass. This was, as mentioned earlier, later confirmed by the discovery of neutrino oscillations [5] [4].
One can then use the exact values of the arrival time for different energies of neutrinos to place an upper bound on the mass of the neutrino. Soon after the event, this upper bound for the electron neutrino was stated as 16 eV/c² [5], while later analysis reduced this to 10 eV/c² [1]. Either way, the mass of the electron neutrino, while certainly non-zero, is inordinately tiny, especially when one considers that the mass of the next lightest particle, the electron, has a mass on the order of 0.5 MeV/c² [16].
The arrival time dispersion also allowed for a calculation of the upper bound on the charge of the neutrino. This is since galactic magnetic fields would deflect charged particles of higher energy less than those of lower energy, meaning the former would arrive sooner [5]. In the event, the differences in arrival times due to this effect was sufficiently tiny to calculate the upper bound of the charge of the neutrino as 3x10⁻¹⁷[5], a charge so miniscule that it is essentially negligible.
SN1987A was a seminal moment in neutrino astrophysics. It not only allowed us to test our theories about core collapse supernovae and demonstrate the effectiveness of our instruments for deep field astrophysics, but once again those same astrophysical observations allowed us to discover more about the physics of the neutrino itself.
Cosmic Bombardment
One of the greatest ongoing mysteries of astrophysics is the origin of so-called Cosmic Ray Particles, typically protons [17], which bombard the upper atmosphere with energies up to Exa-Electron Volts. It is thought that the highest-energy of these particles are of extra-galactic origins [18], but it is very difficult to tie down the exactorigins of these particles since, as charged particles, they are deflected by the electromagnetic fields permeating the cosmos, preventing easy association with a source [9].
Fortunately, neutrinos provide a solution. It is theorised that the acceleration of cosmic ray particles to their extraordinarily high energies can also form ultra-high-energy neutrinos. This occurs when the accelerated protons collide with other matter in the vicinity of the acceleration site, forming a type of meson known as a pions. These can be either charged or neutral, and the neutral pions decay into high-energy gamma rays, while the charged pions decay into neutrino-antineutrino pairs. It is these neutrinos that can be detected on Earth [1].

The IceCube detector, located at the south pole, is one observatory looking for ultra-high-energy neutrinos and, more importantly, their sources. Beginning operation in 2010, by 2015 they had detected 54 neutrinos with energy of the order of 10¹⁵ eV [9]. They had not, however, managed to determine any definite sources for these neutrinos. In 2014, the collaboration published the results of fruitless searches for the sources of ultra-high-energy neutrinos, including detailed studies of 44 specific objects as well as all-sky surveys, none of which showed neutrino fluxes above that of the background [19].
In 2017, however, everything changed. On September 22nd, IceCube detected a 0.29 PeV neutrino and determined its source to be the region of the sky, shown in Figure 4, near the blazar TXS 0505+056.
Blazars are an example of active galactic nuclei (AGN), an artist’s impression of which can be seen in Figure 5. AGN occur when the supermassive black hole at the centre of a galaxy has sufficient material falling onto it that an accretion disk is formed [21]. The extreme electromagnetic fields associated with such a region can accelerate charged particles into near-light speed jets of radiation perpendicular to the plane of the accretion disk [21]. A blazar occurs when one of these jets is pointed directly at the Earth [20].

This particular blazar had been flaring in gamma rays since April of the same year [21], but, since this is fairly common behaviour for such an object, little attention was paid to TXS until after the automated alert issued by IceCube [24]. As well as the gamma-ray observations, which showed a significantly higher gamma ray flux than the background for that region [24], the IceCube collaboration conducted further statistical analysis on their own data from the previous 8 years [21].
They found that there was a neutrino excess associated with the region around TXS, most notably a five-month period from 2014–2015 when 13 ± 5 neutrinos were detected, compared with the background for that region of 5.8 events over a similar time period [21]. They thus concluded that TXS is a ultra-high-energy neutrino source at a significance of 3.5σ [21], which means a 99.87% chance that these emissions are associated with TXS [23]. They also stated that “The coincidence of an IceCube alert with a flaring blazar, combined with a neutrino flare from the same object in archival IceCube data, pinpoints a likely source of high-energy cosmic rays” [21].
A Multi-messenger Future
The detection of ultra-high-energy neutrinos from a flaring blazar is an important discovery for several reasons. The first is the provision of experimental evidence for the theories surrounding one of the most mysterious phenomena in astrophysics: that of the origin of high-energy cosmic ray particles.
But it also is a beautiful demonstration of what is known as multi-messenger astronomy. This is the combination of different media to learn more than is possible using just one astronomy, be it using optical, neutrino or even, with recent advances at LIGO and VIRGO, gravitational wave astronomy. With the boundaries of our astrophysical knowledge being pushed back ever further into the realms of the distant and ultra-high-energy, the future of astronomy is surely multi-messenger.
But, we should not forget the power of neutrino astrophysics on its own. From the early days of solar neutrino observations, to the evidence for core collapse models from SN1987A, and applications beyond those discussed here, such as atmospheric neutrino showers and even the search for proton decay, observations in neutrinos can provide information which cannot otherwise be obtained by optical astronomy.
And of even greater beauty, in my opinion, is the way we have been able to use astrophysical observations to help us learn about the physics of one of the universe’s most elusive particles. The most prominent example of this is the invocation of neutrino oscillations as a solution to the Solar Neutrino Problem and, in turn, the implication that the neutrino must have mass, a divergence from the Standard Model which is, as yet, unexplained. It is these inconsistencies in our models that drive the advancement of physics: who knows where this one will take us?
References
[1] C. Grupen, G. Cowan, T. Stroh and S. Eidekman, Astroparticle Physics, English ed., Springer, 2005.
[2] B. Martin and G. Shaw, “Electron Neutrinos,” in Particle Physics, John Wiley & Sons Ltd, 1992, pp. 24–27.
[3] D. Griffiths, Introduction to Elementary Particles, John Wiley & Sons, 1987.
[4] E. Kearns, T. Kajita and T. Yoji, “Detecting Massive Neutrinos,” Scientific American, vol. 281, no. 2, pp. 66–71, 1999.
[5] J. Bahcall, Neutrino Astrophysics, 1st ed., Cambridge University Press, 1989.
[6] N. E. Holden, T. B. Coplen, J. K. Böhlke, L. V. Tarbox, J. Benefield, J. R. de Laeter, P. G. Mahaffy, G. O’Connor, E. Roth, D. H. Tepper, T. Walczyk, M. E. Weiser and S. Yoneda, “IUPAC Periodic Table the Elements and Isotopes (IPTEI) for the Education Community — Update 2019 (IUPAC Technical Report),” Pure and Applied Chemistry, vol. 90, no. 12, pp. 1823–2092, 2019.
[7] Y. Suzuki, “Solar Neutrinos,” International Journal of Modern Physics A, vol. 15, pp. 201–228, 2000.
[8] N. Jelley, A. McDonald and R. Hamish Robertson, “The Sudbury Neutrino Observatory,” Annual Review of Nuclear and Particle Science, vol. 59, pp. 431–469, 2009.
[9] F. Halzen, “Neutrinos at the Ends of the Earth,” Scientific American, vol. 313, no. 4, pp. 58–63, 2015.
[10] L. Bergström and A. Goobar, “The Role of Neutrinos,” in Cosmology and Particle Astrophysics, 2nd ed., Praxis Publishing Ltd, 2004, pp. 237–272.
[11] C. Moskowitz, “Strange Neutrinos from the Sun Detected for the First Time,” 27 August 2014. [Online]. Available: https://www.scientificamerican.com/article/solar-neutrinos-detected-borexino/.[Accessed 18 November 2019].
[12] J. Bahcall and C. Peña-Garay, “Solar models and solar neutrino oscillations,” New Journal of Physics, vol. 6, 2004.
[13] Q. Ahmed et al., “Measurement of the Rate of interactions produced by 8B at the Sudbury Neutrino Observatory,” Physical Review Letters, vol. 87, no. 7, 2001.
[14] The Nobel Prize, “The Nobel Prize in Physics 2015,” [Online]. Available: https://www.nobelprize.org/prizes/physics/2015/summary/. [Accessed 10 November 2019].
[15] R. West, A. Lauberts, H. Jorgensen and H.-E. Schuster, “Astrometry of SN 1987A and Sanduleak-69 202,” Astronomy and Astrophysics, vol. 177, no. 1–2, pp. L1-L3, 1987.
[16] K. Hirata et al., “Observation in the Kamiokande-II detector of the neutrino burst from supernova SN1987A,” Physical Review D, vol. 38, no. 2, 1988.
[17] NIST, “The NIST Reference on Constants, Units and Uncertainties,” [Online]. Available: https://physics.nist.gov/cgi-bin/cuu/Value?mec2mev|search_for=electron+mass. [Accessed 11 November 2019].
[18] NASA, “Cosmic Rays,” July 2017. [Online]. Available: https://imagine.gsfc.nasa.gov/science/toolbox/cosmic_rays1.html. [Accessed 11 November 2019].
[19] The Pierre Auger Collaboration, “Observation of a large-scale anisotropy in the arrival directions of cosmic rays above 8 × 10¹⁸ eV,” Science, vol. 357, pp. 1266–1270, 2017.
[20] M. Aartsen et al., “Searches for Extended and Point-Like Neutrino Sources with Four Years of IceCube Data,” The Astrophysical Journal, 2014.
[21] R. Blandford, H. Netzer and L. Woltjer, Active Galactic Nuclei, Springer, 1990.
[22] NASA, “Active Galactic Nuclei,” December 2016. [Online]. Available: https://imagine.gsfc.nasa.gov/science/objects/active_galaxies1.html. [Accessed 11 November 2019].
[23] M. Aartsen et al., “Neutrino emission from the direction of the blazar TXS 0506+056 prior to the IceCube-170922A alert,” Science, vol. 361, no. 6398, pp. 147–151, 2018.
[24] M. Aartsen et al., “Multimessenger observations of a flaring blazar coincident with high-energy neutrino IceCube-170922A,” Science, vol. 361, no. 6398, 2018.
[25] R. Evans, “What does a 1-sigma, a 3-sigma or a 5-sigma detection mean?,” 2014. [Online]. Available: https://thecuriousastronomer.wordpress.com/2014/06/26/what-does-a-1-sigma-3-sigma-or-5-sigma-detection-mean/. [Accessed 1 October 2019].
[26] D. Alp et al., “The 30 Year Search for the Compact Object in SN 1987A,” The Astrophysical Jornal, vol. 864, no. 2, 2018.